The Genetic and Evolutionary Drives behind Primate Color Vision
- 1Lions Eye Institute, University of Western Australia, Perth, WA, Australia
- 2Laboratory of Sensory Ecology, Federal University of Rio Grande do Norte, Natal, Brazil
- 3School of Biological Sciences, University of Western Australia, Perth, WA, Australia
- 4Oceans Institute, University of Western Australia, Perth, WA, Australia
Primate color vision is based on two to three cone types in the retina, each expressing a different class of visual pigment, making them the only mammals that possess trichromacy. These pigment classes are the short wavelength-sensitive (SWS1) pigment and the long wavelength-sensitive (LWS) pigment, orthologues of the same pigments found in many other vertebrates, as well as the middle wavelength-sensitive (MWS) pigment, a paralogue to the LWS pigment. Trichromacy was achieved differently in Old World and New World primates. In Old World primates, a duplication of the LWS opsin gene occurred giving rise to a “red-sensitive” or L pigment and a “green-sensitive” or M pigment. Their corresponding L and M genes are adjacent on the X chromosome which, together with their high sequence homology, is the underlying cause for the high frequency of red-green color blindness seen in humans. In New World primates and prosimians, however, the mechanism leading to trichromacy, with one exception, is based on a single polymorphic LWS gene, from which different allelic variants encode pigments with differing spectral peaks. X chromosome inactivation limits expression to just one gene per photoreceptor meaning that trichromacy is only seen in females; while all male are red-green color blind. Despite several leading hypotheses, the reasons for the different evolutionary paths taken by Old and New World primates for trichromacy are still unclear and remain to be confirmed.
Introduction
Color vision arose in early agnathan vertebrates over 540 mya (Xian-Guang et al., 2002; Shu et al., 2003) as a tetrachomatic system based on the presence of four spectrally different types of cone photoreceptors (Collin et al., 2003; Davies et al., 2007b). These cones are defined by which type of visual pigment they express that, in turn, are described by their peak absorbance (λmax) as longwave-sensitive (LWS) with λmax 500–570 nm, middlewave-sensitive (MWS or RH2) with λmax 480–530 nm, and shortwave-sensitive, with two pigments, SWS2 with λmax 400–470 nm and SWS1 with λmax 355–445 nm. Located in the vertebrate retina alongside rod photoreceptors, cones are usually responsible for color vision at normal light levels, while rods are responsible for monochromatic vision in dim light, although rods might also play a role in color vision (Blackwell and Blackwell, 1961; Paramei et al., 1998; Freitag and Pessoa, 2012).
Structurally, visual pigments are members of the GPCR family and consist of a polypeptide chain of 340–370 amino acids that forms seven α-helical transmembrane (TM) domains connected by cytoplasmic and luminal loops, plus an extracellular N-terminus and an intracellular C-terminus, the latter containing phosphorylation sites important in opsin deactivation. In all vertebrate opsins, the TM regions form a cavity in which a retinal chromophore is covalently linked to a conserved Lys residue on the opsin protein via a Schiff base (SB). In mammals, the chromophore is invariably 11-cis-retinal derived from vitamin A1; spectral tuning is determined solely by the amino acid sequence of the opsin protein, with specific residues playing a major role in defining the spectral sensitivity of each pigment. In pigments with a λmax value above 385 nm, the SB is protonated, with a negatively-charged residue at site 113 (usually Glu113) acting as a counterion to stabilize the proton of the SB (Nathans, 1990). An unprotonated SB, on the other hand, is found in SWS1 pigments that show ultraviolet-sensitivity with λmax values around 360 nm (Fasick et al., 2002; Hunt and Peichl, 2014).
Orthologues of the four pigment classes found in cone photoreceptors survived the split between the agnathans and gnathostomes and have been retained by most vertebrate classes, although in many cases, one or more pigments have been lost, with the ancestral tetrachromatic color vision being reduced to trichromacy, dichromacy, or monochromacy. Mammals, in general, fall into the dichromacy category, in which RH2 and SWS1 pigments in monotremes (Davies et al., 2007a; Wakefield et al., 2008), or RH2 and SWS2 pigments in marsupials and placental mammals (Jacobs, 1993), have become non-functional.
Evolution of Color Vision in Primates
It is assumed that at the base of the primate lineage, the ancestral species possessed only the SWS1 and LWS pigments and were dichromats (Hunt et al., 1998). The trichromacy seen in some primate species has been achieved therefore not by the retention of SWS2 or RH2 pigments found in other vertebrate groups but by a duplication of the LWS pigment. This duplication allowed for a mutational drift between the two copies generating two spectrally distinct isoforms maximally sensitive at around 530 nm (M pigment) or 560 nm (L pigment). Interestingly, the three major primate groups, prosimians, New World primates and Old World primates achieved trichromacy via different molecular mechanisms after the New World and Old World landmasses split during the Middle Cretaceous around 65 million years ago (Kious and Tilling, 1994).
Trichromacy in Old World Primates
Amongst mammals, primates are the only ones that possess true trichromatic color vision. In Old World primates, or catarrhines, from Africa and Asia (including humans), this was achieved by a ~40 kb duplication of the X-linked LWS gene that is thought to have occurred at the base of the Old World primate lineage (Nathans et al., 1986b; Hunt et al., 1998; Dulai et al., 1999). Part of this duplicated region comprises the entire coding regions of the LWS opsin gene (Hanna et al., 1997; Dulai et al., 1999), giving rise to a head-to-tail tandem array of LWS genes (Figure 1). The duplicated LWS genes have subsequently diverged to give an upstream copy (OPN1LW) encoding a long wavelength-sensitive (LWS) pigment with λmax around 560 nm and a downstream copy (OPN1MW) encoding a middle wavelength-sensitive (M) pigment with λmax around 535 nm. Trichromacy is therefore achieved by individual cones expressing just one copy of either the L or M pigments together with S cones expressing the autosomal SWS1 gene (OPN1SW). The coding regions of L and M opsin genes have a 98% homology compared to only 40% with the S opsin gene sequence; this is largely the result of the original duplication but maintained by processes, such as gene conversion (Zhou and Li, 1996; Zhao et al., 1998; Hiwatashi et al., 2011). This high level of identity also extends to the introns.
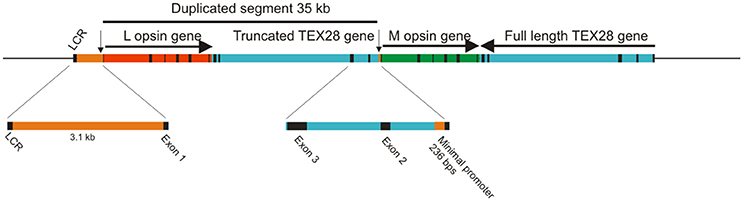
Figure 1. Origin of opsin gene duplication on the X chromosome of Old World primates. The exons for the L and M opsin genes and for the TEX28 gene are shown as black bars. Arrows indicate the direction of transcription. The positions of the upstream LCR and the minimal promoters for the L and M opsin genes (vertical arrows) are shown.
Since the duplicated opsin gene array is present on the X chromosome, the process of X chromosome inactivation (Lyon, 1974) in females is necessary to guarantee that only one array is active in any given cone cell. However, a second mechanism must also be present to ensure that only one gene, either L or M, is expressed, since the gene array is comprised of a single L pigment gene followed by one or more M pigment genes. This is thought to be the role of the locus control region (LCR) located, in humans, between 3.1 and 3.7 kb upstream of the opsin gene array. The LCR is a highly conserved region across vertebrates and acts as a regulatory and enhancer element controlling expression of linked genes. It is not only present in the Old World primate species but also in species where only a single X-linked opsin gene is present (Dulai et al., 1999). Gene activation and regulation is nonetheless thought to require interaction between the LCR and the promoter region immediately upstream of the coding region and that the distance between the two influences the frequency of promoter activation (Shaaban and Deeb, 1998). Since each L and M genes possess a minimal promoter immediately upstream of exon 1, it is thought that the LCR interacts with only one of these gene-specific promoter regions, thereby activating only the downstream gene (Shaaban and Deeb, 1998). Indeed, in a study where transgenic mice carried the human LCR and L and M promoters driving different reporter genes (Smallwood et al., 2002), expression from the proximal L promoter was higher than from the distal M promoter (Figure 2A), and the addition of a 9 kb spacer between the L and M genes resulted in a further decrease in M promoter expression, whereas an LCR duplication resulted in a more equal expression from both promoters. In contrast, placement of the M promoter upstream of the L promoter resulted in a total lack of expression from the L promoter, indicating that distance of the L and M promoters from the LCR affects their level of activity and that the M promoter is the stronger of the two promoters. This may be therefore the mechanism whereby the M promoter compensates for its greater distance from the LCR. The interaction of the LCR with either the L or M promoter is considered to be a stochastic process (Figure 2B); differing strengths of promoter interaction may explain the different L and M cones ratios seen in the retinae of different human individuals that range from a 4:1 ratio in most individuals to a 30:1 ratio in some rare cases (Carroll et al., 2002). In a number of Old World monkey species, however, a 1:1 ratio was found (Bowmaker et al., 1991), and this may again be attributable to the more equal strengths of the respective L and M opsin gene promoters.
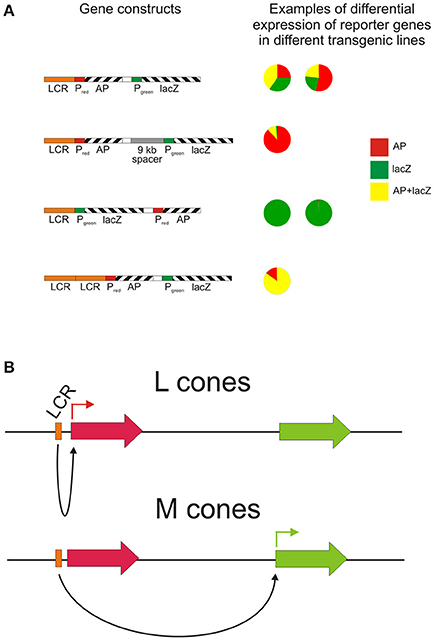
Figure 2. The role of the LCR in directing reporter gene expression from L and M opsin gene promoters. (A) The relative activity of the L and M promoters in four different transgene arrays assessed in a number of transgenic mouse lines by scoring individual cone cells for the expression of the reporter genes. PL, human L opsin gene promoter; PM, human M opsin gene promoter; AP, human placental alkaline phosphatase; lacZ, E. coli β-galactosidase. Re-drawn from Smallwood et al. (2002). (B) Potential mechanism for the stochastic expression of L or M opsin genes via LCR-opsin gene promoter interaction.
The extremely close sequence homology between the L and M pigment genes in primates (Nathans et al., 1986b) has allowed the spectral shifts between the two pigments to be largely driven by substitutions at three amino acid sites, 180 (found in exon 3) and 277 and 285 (both found in exon 5) (Neitz et al., 1991). Amongst the catarrhines, the polar residues Ser, Tyr, and Thr are present at these sites in the L variants of the LWS pigment, respectively, whereas, the non-polar residues Ala, Phe, and Ala are present in the M variants, respectively (Nathans et al., 1986b; Ibbotson et al., 1992). These amino acid differences account for most of the spectral separation of the L pigment at 560 nm and the M pigment at 535 nm and are mostly conserved across Old World primates, including humans (Ibbotson et al., 1992; Dulai et al., 1994). Site 180 is polymorphic in humans; Ser180 is the more common residue in the L pigment but Ala180 is present at a significant frequency to give a slight short wavelength-shift in the L pigment of between 2.6 and 4.3 nm in some individuals (Sanocki et al., 1993).
The close proximity of the L and M genes and their close sequence homology is thought to promote mispairing during meiosis. Where this has been followed by intragenic and intergenic crossing-over within and between the L and M genes in the array, gene loss, gene duplication, and the production of hybrid genes has resulted; it is these latter two events that are responsible for the high frequency of red-green color vision deficiencies in humans (Nathans et al., 1986a; Neitz and Neitz, 2000), and the presence of extra downstream copies of M genes in the array, with copy numbers ranging from one to five and a modal value of two (Nathans et al., 1986a; Drummond-Borg et al., 1989; Feil et al., 1990; Jorgensen et al., 1990). As mentioned above, the L and M gene array is present in an essentially identical form throughout Old World primates, yet red-green color vision deficiencies are extremely rare in non-human primates; genetic defects in the opsin gene array have been detected in just two studies (Jacobs and Williams, 2001; Verrelli et al., 2008). In the first, three male monkeys were found to have a hybrid L/M gene in a colony of 744 male long-tailed macaques (Onishi et al., 1999, 2002), giving a frequency of anomalous trichromacy of only 0.4%, and in the second, an L/M hybrid was identified in a single animal amongst 58 male chimpanzees (Terao et al., 2005); the protanomaly in this animal was confirmed by behavioral tests (Saito et al., 2003). Multiple M opsin genes have been identified in gibbons, with an average incidence of 23.5% across all species studied (Hiwatashi et al., 2011). However, no cases of gene loss from the array or the presence of hybrid genes were noted in 152 individual animals genotyped. Overall therefore, there would appear to be strong evidence that red-green color vision deficiencies are highly detrimental to survival within non-human Old World primate communities and are removed by natural selection.
Trichromacy in New World Primates
The genetic mechanism underlying trichromacy in New World primates, or platyrrhines from Central and South America, is, with one exception, not based on a duplication event, but on the presence of polymorphic copies of the LWS gene (Neitz et al., 1991; Williams et al., 1992), with the different allelic copies giving rise to pigments with λmax values ranging from 535 to 565 nm (Table 1). Since only females can have two X chromosomes, full trichromacy is limited to heterozygous females with different allelic forms of the gene on each chromosome, while all males are dichromats (Mollon et al., 1984). The only exception is found in the howler monkey, Alouatta spp. where full trichromacy in present in both sexes. This is due to a similar, but independent, duplication of the LWS gene as seen in Old World primates (Jacobs et al., 1996a; Dulai et al., 1999), giving rise to separate L and M genes encoding pigments with λmax values of 530 and 558 nm, respectively (Saito et al., 2004; Silveira et al., 2014). This duplication is limited to the Alouatta genus and thought to have happened more recently than the catarrhine event, as demonstrated by the lower rate of substitutions of only 2.7% between the L and M genes (Hunt et al., 1998). The presence of a separate LCR upstream of each opsin gene, indicating that the duplication included both the opsin gene and its upstream LCR (Dulai et al., 1999). The spectral tuning of the howler monkey's L/M pigments however does parallel the mechanism seen in the catarrhine monkeys with identical substitution in sites 180, 277, and 285 defining the λmax values of the L and M pigments (Saito et al., 2004).
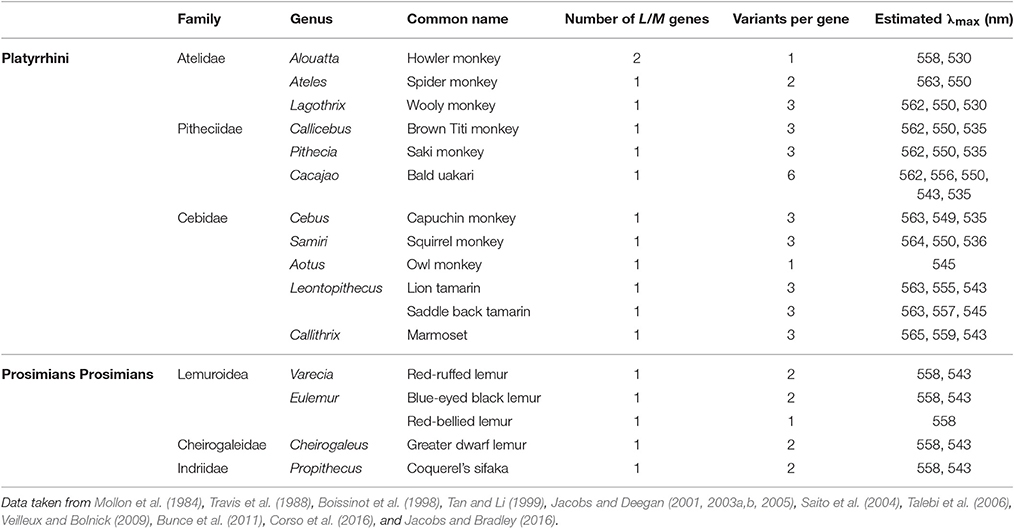
Table 1. The allelic variation found in the LWS/MWS (L/M cone opsin) genes of New World monkeys and prosimians.
Apart from the trichromacy in the howler monkey, most other New World monkeys seem to have evolved along two lines and this split is particularly noticeable between the two major subfamilies of the Cebidea family, the Cebinae and the Callitrichinae. In the Cebinae family, which includes the capuchin and squirrel monkeys, all three sites, 180, 277 and 285, are polymorphic with either Ser/Ala, Tyr/Phe, and Thr/Ala present, respectively (Table 1). The triad Ser-Tyr-Thr accounts for a λmax of 563 nm, Ala-Phe-Thr for a λmax of 550 nm and Ala-Phe-Ala for a λmax of 535 nm. In contrast, in the family Callitrichinae, which includes marmosets and tamarins, site 277 is not polymorphic with only Tyr found, so the λmax values for L and M pigments differ by a maximum of only 19–20 nm. In this family branch the triad combination and respective λmax are as follows: Ser-Tyr-Thr for 563, Ala-Tyr-Thr for 556, and Ala-Tyr-Ala for 543 nm. However, it is important to notice that, in many cases, different combinations of residues across the three sites (Table 1) can produce three or more different spectral variants with intermediate λmax values (Jacobs and Deegan, 2003a). In the other two families that form the New World monkey parvorder, Atelidae, and Pitheciidae (Perelman et al., 2011), trichromacy appears to have followed the same evolutionary process seen in the Cebinae family (Riba-Hernandez et al., 2004); however, the bald uakari (C. calvus) found in the Pitheciidae family, has recently been reported to have six functional polymorphic L opsin alleles with different combinations of residues at sites 180, 277 and 285, although two of these are estimated to have the λmax at 550 nm (Corso et al., 2016).
Trichromacy in Prosimians
Polymorphism is also the mechanism of trichromacy in prosimians (Tan and Li, 1999), the lorises and galagos from Africa and Asia, the lemurs from Madagascar and the tarsiers from Southeast Asia, which form the most basal branch of the primate phylogeny. A polymorphic LWS gene has been reported in three diurnal prosimian species, the red ruffed lemur, Varecia variegata rubra, Coquerel's sifaka, Propithecus verreauxi coquereli, and the blue-eyed black lemur, Eulemur macaco flavifrons, and in a nocturnal species, the greater dwarf lemur, Cheirogaleus major (Tan and Li, 1999; Jacobs et al., 2002; Veilleux and Bolnick, 2009). However, in these species, the spectral shifts between pigments arise from substitution at just site 285, with Thr present in the L pigment gene and Ala in the M pigment gene, encoding pigments with λmax of 558 and 543–545 nm, respectively (Tan and Li, 1999; Jacobs et al., 2002; Veilleux and Bolnick, 2009). In the majority of non-polymorphic species, an M pigment (with Ala285) is present, with L pigments (with Thr285) found in only three species. Sites 180 and 277 seem so far to be conserved across all prosimians with Ala and Tyr present, respectively (Tan and Li, 1999). Although polymorphism has been reported in just four species, it is possible that this may be an underestimate of the number of trichromatic prosimian species since many of the studies have been restricted to small cohorts of animals. However, a recent study using a large sample group of the red-bellied lemurs (Eulemur rubriventer) showed that this species has a 100% frequency of the L allele with Thr at site 285, probably due to a genetic bottle neck event in the studied population (Jacobs and Bradley, 2016). Indeed this same group later demonstrated that amongst lemur species, the presence of M or L pigments is highly variable (Jacobs et al., 2016).
The presence of trichromacy mediated by the same mechanism of polymorphic LWS genes in New World monkeys and different present-day species of the separate prosimian branches of tarsiers and strepsirhines suggests that the ancestral primate may have been a trichromat with polymorphic LWS genes (Melin et al., 2013b). Indeed when comparing the sequences of primate LWS genes, including several prosimian species, the most parsimonious conclusion is that the ancestral primate carried polymorphic copies of the LWS gene (Tan et al., 2005).
Spectral Tuning of the SWS1 Pigment
The SWS1 opsin is subdivided into two categories; ultraviolet sensitive (UVS), with λmax < 390 nm (355–390 nm), and violet sensitive (VS), with λmax > 390 nm (390–450 nm). It is found throughout all vertebrate groups. The UVS SWS1 pigments almost certainly represent the ancestral form of the pigment (Hunt et al., 2004, 2009) and within mammals, they are relatively common in marsupials but are restricted in eutherians to just a sub-set of species from the Orders Rodentia, Chiroptera, and Insectivora (Hunt and Peichl, 2014). In mammalian SWS1 pigments, the tuning mechanism between UVS and VS pigments is defined by site 86. Substitutions at this site have been shown to produce complete shifts from VS to UVS and vice-versa in several species. Phe86 is generally associated with a UVS pigment (Hunt et al., 2004) and it is the replacement of this residue by either Tyr, Ser, or Val (Cowing et al., 2002; Parry et al., 2004; Yokoyama et al., 2005) that is responsible for the loss of UVS in a number of non-primate species (Hunt et al., 2007).
In primates, all species possess a violet-sensitive (VS) pigment (Bowmaker et al., 1991; Hunt et al., 1995) but the tuning method used to change from the ancestral UV state to current violet sensitivity remains uncertain. It was initially proposed from mutagenesis studies of the mouse UVS pigment, that tuning from UV to violet requires the simultaneous replacement of residues at three sites, Phe86Leu, Thr93Pro, and Ser118Thr (Yokoyama and Shi, 2000; Shi et al., 2001). However, other residue changes at these sites are found in some species of New World primates and prosimians, which all seem to have a VS SWS1 pigment (Figure 3). Interestingly, Phe86 is present in one species, the aye-aye, a prosimian endemic to Madagascar (Carvalho et al., 2012), but expression of the aye-aye pigment showed peak sensitivity in the violet region at 409 nm (Carvalho et al., 2012). This eliminated the possibility of a major role of Phe86 in conferring UVS in primate pigments. Analysis of several primate SWS1 sequences showed that the only residue that is consistently conserved in primate VS pigments is Pro93. Since Pro93 is also found in the VS pigment of the clawed frog (Starace and Knox, 1998), it is not surprising that when a Pro93Thr substitution was introduced into the aye-aye pigment, it was capable of causing a dramatic shortwave shift of 38 nm, resulting in a UVS pigment with λmaxof 371 nm (Carvalho et al., 2012). This does not however rule out a role for site 86 in the initial shift to violet sensitivity at the base of the primate lineage, especially since Tyr86 is found in the SWS1 gene of the tree shrew and colugo (Moritz et al., 2013), close relatives of the primates. The most parsimonious scenario is that Phe86 was present at the base of the primate lineage but underwent a series of substitutions in the various primate lineages where replacement of Phe by either Leu, Tyr, Asn, Val, Ser, or Cys occurred (Carvalho et al., 2012). A subset of these changes (Ser, Tyr, and Val) have been shown to generate shifts into the violet region (Hunt et al., 2007) but if a Thr93 Pro substitution also occurred at the base of the primates, this might have removed constraints on the residue at site 86, thereby allowing different substitutions at this site (Carvalho et al., 2012).
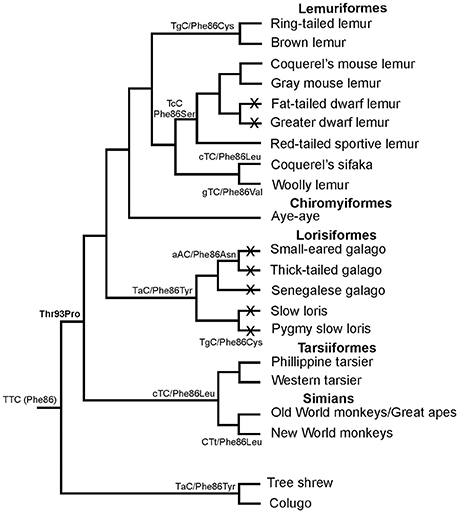
Figure 3. Phylogenetic relationships of different primate species showing the different residues found at site 86 in S cone opsins, together with the corresponding codon sequences. Under the scenario presented, Phe86 was present in the ancestral primate and retained by the aye-aye. Replacement of Phe by either Leu, Cys, Asn, Val, Ser, or Cys then occurred by single nucleotide changes at each step to generate the amino acid changes. Crosses on lineages indicate species where SWS1 pseudogenes are present. Based on data from Carvalho et al. (2012).
Monochromacy in Primates
The basic requirement for color vision is the presence of at least two spectrally distinct cone types in the retina. Most mammals possess a dichromatic color system, so the loss of one spectral cone type is thought to preclude cone-based color vision. Within mammals, the loss of the S cones seems to be the rule in most marine species but has also been observed in a few terrestrial species, with examples in most major mammalian groups like carnivores, rodents and primates (Peichl and Moutairou, 1998; Peichl et al., 2001; Newman and Robinson, 2005; Jacobs, 2013). In many of these species, a SWS1 opsin pseudogene is still present in the genome (Peichl, 2005). In the primate group (Table 2), the owl monkey, and several species of lorises, galagos and tarsiers (part of the prosimian branch) have been shown to carry a SWS1 pseudogene (Jacobs et al., 1996b; Kawamura and Kubotera, 2004; Tan et al., 2005); since these species do not possess a polymorphic or duplicated LWS gene, they are considered to have monochromatic vision (Jacobs et al., 1993, 1996b; Tan and Li, 1999; Levenson et al., 2007; Mundy et al., 2016).
It was initially thought that the last common ancestor of extant primates was nocturnal since this trait was carried on in most extant species of prosimians. Since the loss of a functional SWS1 pigment amongst terrestrial mammals is restricted to nocturnal species, it is not surprising that the only primates to have lost the SWS1 pigment are also nocturnal (Jacobs, 2013). However, the nocturnal ancestral primate hypothesis has been challenged by a study that evaluated the selective constraints on the SWS1 gene across several species of prosimians (Tan et al., 2005). They were able to demonstrate that in prosimians carrying a non-functional S opsin gene, different levels of functional relaxation were found in the three major prosimian clades (tarsiers, lemurs and bushbabies/lorises) where each one accumulated completely different mutations generating separate pseudogenes. This indicates that nocturnality, and subsequent loss of the SWS1 gene, most likely occurred as separate events in each prosimian clade, rejecting the claim that the ancestral primate was nocturnal (Tan et al., 2005).
The only other nocturnal primates are found in the Aotus genus of the platyrrhine monkeys. They have been shown to possess, in addition to the rod pigment, only an M cone pigment with λmax of 543 nm (Jacobs et al., 1993). A number of nucleotide deletions, insertions and nonsense mutations were found in the SWS1 gene in three species of Aotus, rendering it non-functional (Jacobs et al., 1996b; Levenson et al., 2007), that seem to have occurred at the base of the lineage. Interestingly, although most Aotus species are considered strictly nocturnal (Figure 4), one of the three species analyzed, A. azarai, displays a cathemeral activity pattern (active both during daylight and night time) (Fernandez-Duque et al., 2010). Since the phylogenetic analysis strongly implies that the loss of the SWS1 gene occurred at the base of this genus, this shift to a cathemeral lifestyle would have been acquired after these species diverged; monochromatic vision does not appear therefore to have hindered this shift in lifestyle.
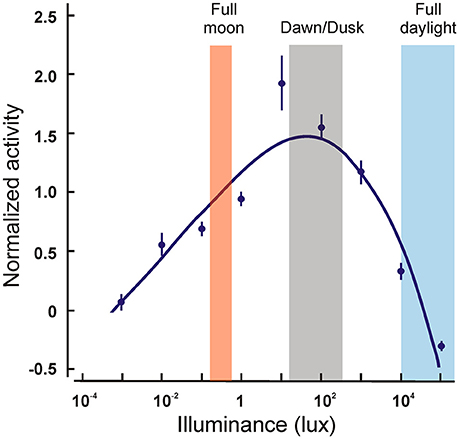
Figure 4. Locomotor activity levels of wild Aotus azarai as a function of ambient illuminance levels. The points represent the averaged activity levels for nine animals. Note that the greatest activity occurs at dawn and dusk, and that some activity is still present at very dim or at very bright light levels. Data replotted from Fernandez-Duque et al. (2010).
Evolutionary Drives Behind Trichromacy in Primates
The diversity of color vision phenotypes found amongst primates has stimulated a prolific discussion over the past three decades about the implications of color vision for primate foraging behaviors, predation avoidance, social behavior, mate choice, and group dynamics (Figure 5; Buchanan-Smith, 2005). Nevertheless, the selective forces and relative benefits influencing the evolution of color vision in primates are still under debate.
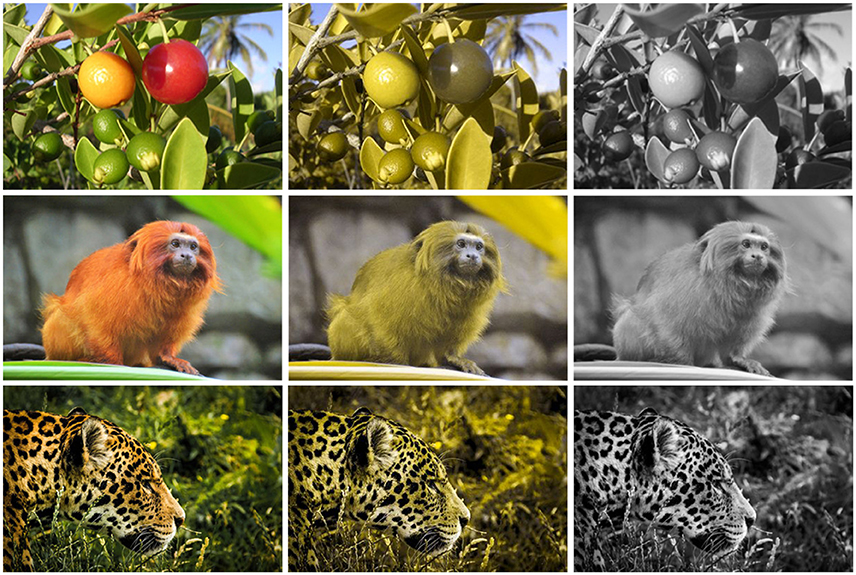
Figure 5. Examples of evolutionary drives that might have influenced the evolution of primate color vision. Original pictures (left column) and simulations of dichromatic (middle column) and monochromatic (right column) vision are shown.
Since the nineteenth century, fruit coloration has been understood as a trait that evolved in parallel with animal color vision (Jacobs, 2007), where the primary advantage of color vision would be to allow the detection of conspicuously colored targets against a green dappled background foliage, in which lightness would vary randomly (Mollon, 1989). In line with this, visual modeling studies have predicted that trichromats should have improved discrimination in the green-red part of the spectrum, since they express two M/L opsins, giving them an advantage for conspicuous fruit detection (Osorio and Vorobyev, 1996; Regan et al., 2001; Perini et al., 2009). Therefore, the poly-allelic X-linked polymorphism of New World primates and prosimians could be seen as resulting from a heterotic process (Mollon et al., 1984). Evidence from behavioral experiments has provided some support for this frugivory hypothesis (Caine and Mundy, 2000; Smith et al., 2003; Leonhardt et al., 2009; Bompas et al., 2013), although indications of a clear trichromatic advantage for fruit foraging under natural conditions (Melin et al., 2009; Veilleux et al., 2016) and of a heterozygote superiority (Fedigan et al., 2014) are still not fully resolved. So how could the frugivory hypothesis explain the difference in color vision found between Old World and New World primates? Apparently, fruits from Africa (Uganda), which darken when ripe, are smaller than South American (French Guiana) fruits that lighten during ripening (Sumner and Mollon, 2000). Since dichromats might enhance their color vision capacities when target size is augmented (Gomes et al., 2005; Melin et al., 2013a), the diet of platyrrhines should be easier to detect against a foliage background, when compared to catarrhines' diet, reducing the selective advantage of trichromacy over dichromacy in New World primates.
Two decades ago, however, the frugivory hypothesis was challenged by an alternative hypothesis (Lucas et al., 1998; Dominy and Lucas, 2001), which proposed that young edible leaves, but not ripe edible fruits, would be systematically selected by trichromatic primates based on their redness (Lucas et al., 2003). Supporters of the folivory hypothesis also suggested that the abundance of cryptically colored keystone fruits in the New World and Madagascar might not favor the evolution of routine trichromacy, since young leaves never became a critical fall-back food in these places, as they did in areas inhabited by Old World primates (Dominy et al., 2003). This hypothesis seems to hold true when we recall that the most folivorous New World primates, the howler monkey (Alouatta spp.), are also the only platyrrhines to express full trichromacy (Jacobs et al., 1996a; Dominy and Lucas, 2001). However, wooly spider monkeys (Brachyteles arachnoides), a platyrrhine with a high leaf content in their diet, have been shown to be a polymorphic species with a relatively low frequency of trichromatic individuals in their populations (Talebi et al., 2006), greatly weakening the folivory hypothesis.
Alternatively, given their inferior performance in relation to trichromats in detecting conspicuous food items, dichromats may compensate their disadvantage by identifying cryptic/camouflaged food (Mollon, 1989) since neural processing of information on color and shape/texture occurs concurrently and competitively (Liebe et al., 2009). Thus, individuals with greater dependence on color cues (e.g., trichromats) would be hindered by chromatic noise, while those with poorer color vision (e.g., dichromats) would benefit by assessing achromatic cues, important for the identification of shapes, outlines and textures (Regan et al., 2001). Several behavioral studies have supported the idea that the visual polymorphism of New World primates balances the advantages of dichromats in recognizing camouflaged objects (Morgan et al., 1992; Saito et al., 2005) or insects (Melin et al., 2007; Smith et al., 2012), and of trichromats in detecting conspicuous food targets (Caine and Mundy, 2000; Smith et al., 2003; Leonhardt et al., 2009; Bompas et al., 2013). In fact, recent molecular evidence has shown that the visual polymorphism of New World primates has been maintained by balancing selection (Hiwatashi et al., 2010). Additionally, it has been shown that under certain circumstances (e.g., lower luminosities), dichromats may improve their color perception (Freitag and Pessoa, 2012), matching the performances of trichromats in conspicuous food detection tasks (Caine et al., 2010).
Unfortunately, progress in our understanding of the evolutionary drive behind trichromacy has been limited by an inordinate focus on a single hypothesis, the importance of trichromacy for food selection, while ignoring other sensory challenges that might impact on fitness. In fact, a few studies suggest that color may not even play an essential role in primate short distance food detection (Hiramatsu et al., 2008, 2009; Bompas et al., 2013), so other selective forces (e.g., detection of predators; sexual selection and social dynamics) should be examined. Color modeling studies have predicted that trichromats should outperform dichromats when discriminating socio-sexual signals (Sumner and Mollon, 2003; Changizi et al., 2006) and predators (Pessoa et al., 2014). The influence of color vision on sexual selection and social dynamics have been studied in Old World primates (Waitt et al., 2003; Changizi et al., 2006; Setchell et al., 2009; Gerald et al., 2010) and, to a lesser extent, in prosimians (Clough et al., 2009) and New World primates (Smith et al., 2005; Surridge et al., 2005; Moreira et al., 2015). However, many studies may have overestimated animal color variation, by using subjective human perception when investigating other primate socio-reproductive signals (Higham et al., 2010). The few visual modeling studies that have considered the visual system of certain primate species indicate that chromatic cues might not be essential for socio-reproductive signaling, and that dichromacy should be sufficient (Higham et al., 2010; Moreira et al., 2015).
Since color can either be used to camouflage an object from its background, through chromatic noise, or improve visual recognition of a target (Li and Lennie, 2001), two non-excluding hypotheses might be drawn in relation to the advantages and disadvantages of color vision to camouflaged predator detection. In other words, the color pattern of some predators (e.g., yellow black spotted cats) may interact with the background pattern of dappled green foliage in a way that produces chromatic noise and only allows predator identification by achromatic cues, favoring dichromats. On the other hand, the same yellow-coated predators may also contrast with the green foliage background, enabling their detection by chromatic information, favoring trichromats. Through color vision modeling and behavioral experiments, the only study to test the predator detection hypothesis to date demonstrated that trichromats excel over dichromats when searching for camouflaged predators (Pessoa et al., 2014). This study suggests that the diversity and distribution of color vision in extant anthropoid taxa could reflect the selective advantages of trichromats and dichromats in detecting predators (Pessoa et al., 2014) and cryptic insects (Melin et al., 2007), respectively. The color vision polymorphism of New World primates would be expected therefore to have developed in small anthropoids, since these small bodied primates tend to include more insects and fewer leaves in their diets (Fleagle, 1999). Predation pressure is also much higher (Stanford, 2002), so the presence of both trichromatic and dichromatic individuals may be beneficial. Howler monkeys (Alouatta spp.), on the other hand, that are folivorous and do not rely on insects, are subjected to a disproportionately high predation risk (Terborgh, 1983; Calleia et al., 2009) that might have selected for their full trichromacy, without the need for unnecessary dichromatic individuals.
Conclusion
In primates, trichromacy arose from an ancestral dichromatic state. In Old World (catarrhine) primates, this was achieved through a gene duplication that expanded the L/M array on the X chromosome. By contrast, trichromacy in New World (platyrrhine) primates and prosimians (with the exception of the howler monkey that evolved a similar mechanism to the catarrhines) is mediated by allelic variants of the L cone opsin gene that encodes spectrally distinct photopigments.
All primates express an SWS1 photopigment that is VS and in all cases except one, the residue present at site 86 is consistent with a VS pigment. The one exception is the pigment in the prosimian aye-aye where Phe86 is present; the presence of Phe at site 86 confers UVS in all non-avian vertebrates but in the aye-aye, the pigment remains VS but with a spectral peak short-wavelength shifted to a value that is near the UVS/VS boundary. In other species, such as the owl monkey and a number of prosimians, the SWS1 gene has become pseudogenized; these species are therefore considered to be cone monochromats. Thus, it is possible that the single cone type in these species has been conserved for achromatic discrimination. It is also possible that under mesopic (twilight) conditions, where both rod and cones are active, a state of rudimentary or conditional dichromacy exists.
The nature of the selection pressures and advantages (or disadvantages) behind the evolution of trichromatic vision in primates continues to be the subject of debate, with projected roles in the foraging of ripe fruit, the detection of more nutritious young leaves, and in the breaking of predator camouflage. As yet however, no single hypothesis has been shown to explain convincingly the evolution and conservation of trichromacy in all cases, so it may be that different combinations all these factors may be active in different species.
Author Contributions
DH was responsible along with WD for conceiving the review and in outlining the initial contents. This was subsequently extended and modified by DH, LC, and DP. DH prepared the early drafts and the final submitted version, as well as Figures 1–4. LC was responsible for extending the section of the review that covers primate genetics, DP with the section of the review that covers evolutionary drive, plus Figure 5, and JM with the section of the review that deals with human color vision defects. All authors were involved in the final editing and review of the paper.
Funding
This work was supported by an ARC Discovery Early Career Research Award (DE140100320) to LC, an ARC Discovery Project grant (DP140102117) awarded to WD and DH, and an ARC Future Fellowship (FT110100176) held by WD.
Conflict of Interest Statement
The authors declare that the research was conducted in the absence of any commercial or financial relationships that could be construed as a potential conflict of interest.
References
Blackwell, H. R., and Blackwell, O. M. (1961). Rod and cone receptor mechanisms in typical and atypical congenital achromatopsia. Vis. Res. 1, 62–107. doi: 10.1016/0042-6989(61)90022-0
Boissinot, S., Tan, Y., Shyue, S. K., Schneider, H., Sampaio, I., Neiswanger, K., et al. (1998). Origins and antiquity of X-linked triallelic color vision systems in New World monkeys. Proc. Natl. Acad. Sci. U.S.A. 95, 13749–13754. doi: 10.1073/pnas.95.23.13749
Bompas, A., Kendall, G., and Sumner, P. (2013). Spotting fruit versus picking fruit as the selective advantage of human colour vision. Iperception 4, 84–94. doi: 10.1068/i0564
Bowmaker, J. K., Astell, S., Hunt, D. M., and Mollon, J. D. (1991). Photosensitive and photostable pigments in the retinae of old world monkeys. J. Exp. Biol. 156, 1–19.
Buchanan-Smith, H. M. (2005). Recent advances in color vision research. Am. J. Primatol. 67, 393–398. doi: 10.1002/ajp.20194
Bunce, J. A., Isbell, L. A., Neitz, M., Bonci, D., Surridge, A. K., Jacobs, G. H., et al. (2011). Characterization of opsin gene alleles affecting color vision in a wild population of titi monkeys (Callicebus brunneus). Am. J. Primatol. 73, 189–196. doi: 10.1002/ajp.20890
Caine, N. G., and Mundy, N. I. (2000). Demonstration of a foraging advantage for trichromatic marmosets (Callithrix geoffroyi) dependent on food colour. Proc. Biol. Sci. 267, 439–444. doi: 10.1098/rspb.2000.1019
Caine, N. G., Osorio, D., and Mundy, N. I. (2010). A foraging advantage for dichromatic marmosets (Callithrix geoffroyi) at low light intensity. Biol. Lett. 6, 36–38. doi: 10.1098/rsbl.2009.0591
Calleia, F. O., Rohe, F., and Gordo, M. (2009). Hunting strategy of the margay (Leopardus wiedii) to attract the wild pied tamarin (Saguinus bicolor). Neotrop. Primates 16, 32–34. doi: 10.1896/044.016.0107
Carroll, J., Neitz, J., and Neitz, M. (2002). Estimates of L:M cone ratio from ERG flicker photometry and genetics. J. Vis. 2, 531–542. doi: 10.1167/2.8.1
Carvalho, L. S., Davies, W. L., Robinson, P. R., and Hunt, D. M. (2012). Spectral tuning and evolution of primate short-wavelength-sensitive visual pigments. Proc. Biol. Sci. 279, 387–393. doi: 10.1098/rspb.2011.0782
Changizi, M. A., Zhang, Q., and Shimojo, S. (2006). Bare skin, blood and the evolution of primate colour vision. Biol. Lett. 2, 217–221. doi: 10.1098/rsbl.2006.0440
Clough, D., Heistermann, M., and Kappeler, P. M. (2009). Individual facial coloration in male eulemur fulvus rufus: a condition-dependent ornament? Int. J. Primatol. 30, 859–875. doi: 10.1007/s10764-009-9379-5
Collin, S. P., Knight, M. A., Davies, W. L., Potter, I. C., Hunt, D. M., and Trezise, A. E. (2003). Ancient colour vision: multiple opsin genes in the ancestral vertebrates. Curr. Biol. 13, R864–R865. doi: 10.1016/j.cub.2003.10.044
Corso, J., Bowler, M., Heymann, E. W., Roos, C., and Mundy, N. I. (2016). Highly polymorphic colour vision in a New World monkey with red facial skin, the bald uakari (Cacajao calvus). Proc. Biol. Sci. 283:20160067. doi: 10.1098/rspb.2016.0067
Cowing, J. A., Poopalasundaram, S., Wilkie, S. E., Robinson, P. R., Bowmaker, J. K., and Hunt, D. M. (2002). The molecular mechanism for the spectral shifts between vertebrate ultraviolet- and violet-sensitive cone visual pigments. Biochem. J. 367, 129–135. doi: 10.1042/bj20020483
Davies, W. L., Carvalho, L. S., Cowing, J. A., Beazley, L. D., Hunt, D. M., and Arrese, C. A. (2007a). Visual pigments of the platypus: a novel route to mammalian colour vision. Curr. Biol. 17, R161–R163. doi: 10.1016/j.cub.2007.01.037
Davies, W. L., Cowing, J. A., Carvalho, L. S., Potter, I. C., Trezise, A. E., Hunt, D. M., et al. (2007b). Functional characterisation and regulation of visual pigment gene expression in an anadromous lamprey. FASEB J. 21, 2713–2724. doi: 10.1096/fj.06-8057com
Deegan, J. F. I., and Jacobs, G. H. (1996). Spectral sensitivity and photopigmetns of a nocturnal prosimian, the bushbaby (Otolemur crassicaudata). Am. J. Primatol. 40, 55-66.
Dominy, N. J., and Lucas, P. W. (2001). Ecological importance of trichromatic vision to primates. Nature 410, 363–366. doi: 10.1038/35066567
Dominy, N. J., Svenning, J. C., and Li, W. H. (2003). Historical contingency in the evolution of primate color vision. J. Hum. Evol. 44, 25–45. doi: 10.1016/S0047-2484(02)00167-7
Drummond-Borg, M., Deeb, S. S., and Motulsky, A. G. (1989). Molecular patterns of X chromosome-linked color vision genes among 134 men of European ancestry. Proc. Natl. Acad. Sci. U.S.A. 86, 983–987. doi: 10.1073/pnas.86.3.983
Dulai, K. S., Bowmaker, J. K., Mollon, J. D., and Hunt, D. M. (1994). Sequence divergence, polymorphism and evolution of the middle-wave and long-wave visual pigment genes of great apes and Old World monkeys. Vis. Res. 34, 2483–2491. doi: 10.1016/0042-6989(94)90233-X
Dulai, K. S., Von Dornum, M., Mollon, J. D., and Hunt, D. M. (1999). The evolution of trichromatic color vision by opsin gene duplication in new world and old world primates. Genome Res. 9, 629–638.
Fasick, J. I., Applebury, M. L., and Oprian, D. D. (2002). Spectral tuning in the mammalian short-wavelength sensitive cone pigments. Biochemistry 41, 6860–6865. doi: 10.1021/bi0200413
Fedigan, L. M., Melin, A. D., Addicott, J. F., and Kawamura, S. (2014). The heterozygote superiority hypothesis for polymorphic color vision is not supported by long-term fitness data from wild neotropical monkeys. PLoS ONE 9:e84872. doi: 10.1371/journal.pone.0084872
Feil, R., Aubourg, P., Heilig, R., and Mandel, J. L. (1990). A 195-kb cosmid walk encompassing the human Xq28 color vision pigment genes. Genomics 6, 367–373. doi: 10.1016/0888-7543(90)90578-I
Fernandez-Duque, E., de la Iglesia, H., and Erkert, H. G. (2010). Moonstruck primates: owl monkeys (Aotus) need moonlight for nocturnal activity in their natural environment. PLoS ONE 5:e12572. doi: 10.1371/journal.pone.0012572
Freitag, F. B., and Pessoa, D. M. (2012). Effect of luminosity on color discrimination of dichromatic marmosets (Callithrix jacchus). J. Opt. Soc. Am. A Opt. Image Sci. Vis. 29, A216–A222. doi: 10.1364/JOSAA.29.00A216
Gerald, M. S., Ayala, J., Ruiz-Lambides, A., Waitt, C., and Weiss, A. (2010). Do females pay attention to secondary sexual coloration in vervet monkeys (Chlorocebus aethiops)? Naturwissenschaften 97, 89–96. doi: 10.1007/s00114-009-0619-5
Gomes, U. R., Pessoa, D. M. A., Suganuma, E., Tomaz, C., and Pessoa, V. F. (2005). Influence of stimuli size on color discrimination in capuchin monkeys. Am. J. Primatol. 67, 437–446. doi: 10.1002/ajp.20198
Hanna, M. C., Platts, J. T., and Kirkness, E. F. (1997). Identification of a gene within the tandem array of red and green color pigment genes. Genomics 43, 384–386. doi: 10.1006/geno.1997.4830
Higham, J. P., Brent, L. J. N., Dubuc, C., Accamando, A. K., Engelhardt, A., Gerald, M. S., et al. (2010). Color signal information content and the eye of the beholder: a case study in the rhesus macaque. Behav. Ecol. 21, 739–746. doi: 10.1093/beheco/arq047
Hiramatsu, C., Melin, A. D., Aureli, F., Schaffner, C. M., Vorobyev, M., and Kawamura, S. (2009). Interplay of olfaction and vision in fruit foraging of spider monkeys. Anim. Behav. 77, 1421–1426. doi: 10.1016/j.anbehav.2009.02.012
Hiramatsu, C., Melin, A. D., Aureli, F., Schaffner, C. M., Vorobyev, M., Matsumoto, Y., et al. (2008). Importance of achromatic contrast in short-range fruit foraging of primates. PLoS ONE 3:e3356. doi: 10.1371/journal.pone.0003356
Hiwatashi, T., Mikami, A., Katsumura, T., Suryobroto, B., Perwitasari-Farajallah, D., Malaivijitnond, S., et al. (2011). Gene conversion and purifying selection shape nucleotide variation in gibbon L/M opsin genes. BMC Evol. Biol. 11:312. doi: 10.1186/1471-2148-11-312
Hiwatashi, T., Okabe, Y., Tsutsui, T., Hiramatsu, C., Melin, A. D., Oota, H., et al. (2010). An explicit signature of balancing selection for color-vision variation in new world monkeys. Mol. Biol. Evol. 27, 453–464. doi: 10.1093/molbev/msp262
Hunt, D. M., Carvalho, L. S., Cowing, J. A., and Davies, W. L. (2009). Evolution and spectral tuning of visual pigments in birds and mammals. Philos. Trans. R Soc. Lond. B Biol. Sci. 364, 2941–2955. doi: 10.1098/rstb.2009.0044
Hunt, D. M., Carvalho, L. S., Cowing, J. A., Parry, J. W., Wilkie, S. E., Davies, W. L., et al. (2007). Spectral tuning of shortwave-sensitive visual pigments in vertebrates. Photochem. Photobiol. 83, 303–310. doi: 10.1562/2006-06-27-IR-952
Hunt, D. M., Cowing, J. A., Patel, R., Appukuttan, B., Bowmaker, J. K., and Mollon, J. D. (1995). Sequence and evolution of the blue cone pigment gene in old and new world primates. Genomics 27, 535–538. doi: 10.1006/geno.1995.1088
Hunt, D. M., Cowing, J. A., Wilkie, S. E., Parry, J., Poopalasundaram, S., and Bowmaker, J. K. (2004). Divergent mechanisms for the tuning of shortwave sensitive visual pigments in vertebrates. Photochem. Photobiol. Sci. 3, 713–720. doi: 10.1039/b314693f
Hunt, D. M., Dulai, K. S., Cowing, J. A., Julliot, C., Mollon, J. D., Bowmaker, J. K., et al. (1998). Molecular evolution of trichromacy in primates. Vis. Res. 38, 3299–3306. doi: 10.1016/S0042-6989(97)00443-4
Hunt, D. M., and Peichl, L. (2014). S cones: evolution, retinal distribution, development and spectral sensitivity. Vis. Neurosci. 31, 115–138. doi: 10.1017/S0952523813000242
Ibbotson, R. E., Hunt, D. M., Bowmaker, J. K., and Mollon, J. D. (1992). Sequence divergence and copy number of the middle- and long-wave photopigment genes in Old World monkeys. Proc. Biol. Sci. 247, 145–154. doi: 10.1098/rspb.1992.0021
Jacobs, G. H., and Deegan, J. F. II. (2001). Photopigments and colour vision in New World monkeys from the family Atelidae. Proc. Biol. Sci. 268, 695–702. doi: 10.1098/rspb.2000.1421
Jacobs, G. H., and Deegan, J. F. II. (2003a). Cone pigment variations in four genera of new world monkeys. Vis. Res. 43, 227–236. doi: 10.1016/S0042-6989(02)00565-5
Jacobs, G. H., and Deegan, J. F. II. (2003b). Diurnality and cone photopigment polymorphism in strepsirrhines: examination of linkage in Lemur catta. Am. J. Phys. Anthropol. 122, 66–72. doi: 10.1002/ajpa.10309
Jacobs, G. H., and Deegan, J. F. II. (2005). Polymorphic New World monkeys with more than three M/L cone types. J. Opt. Soc. Am. A Opt. Image Sci. Vis. 22, 2072–2080. doi: 10.1364/JOSAA.22.002072
Jacobs, G. H., Deegan, J. F. D., Neitz, J., Crognale, M. A., and Neitz, M. (1993). Photopigments and color vision in the nocturnal monkey, Aotus. Vis. Res. 33, 1773–1783. doi: 10.1016/0042-6989(93)90168-V
Jacobs, G. H., Deegan, J. F. II., Tan, Y., and Li, W. H. (2002). Opsin gene and photopigment polymorphism in a prosimian primate. Vis. Res. 42, 11–18. doi: 10.1016/S0042-6989(01)00264-4
Jacobs, G. H., Neitz, M., Deegan, J. F., and Neitz, J. (1996a). Trichromatic colour vision in New World monkeys. Nature 382, 156–158. doi: 10.1038/382156a0
Jacobs, G. H., Neitz, M., and Neitz, J. (1996b). Mutations in S-cone pigment genes and the absence of colour vision in two species of nocturnal primate. Proc. Biol. Sci. 263, 705–710. doi: 10.1098/rspb.1996.0105
Jacobs, G. H., and Williams, G. A. (2001). The prevalence of defective color vision in Old World monkeys and apes. Col. Res. Appl. 26, S123–S127. doi: 10.1002/1520-6378(2001)26:1+<::AID-COL27>3.0.CO;2-6
Jacobs, G. H. (1993). The distribution and nature of colour vision among the mammals. Biol. Rev. 68, 413–471. doi: 10.1111/j.1469-185X.1993.tb00738.x
Jacobs, G. H. (2007). New world monkeys and color. Int. J. Primatol. 28, 729–759. doi: 10.1007/s10764-007-9168-y
Jacobs, G. H. (2013). Losses of functional opsin genes, short-wavelength cone photopigments, and color vision-A significant trend in the evolution of mammalian vision. Vis. Neurosci. 30, 39–53. doi: 10.1017/S0952523812000429
Jacobs, R. L., and Bradley, B. J. (2016). Considering the influence of nonadaptive evolution on primate color vision. PLoS ONE 11:e0149664. doi: 10.1371/journal.pone.0149664
Jacobs, R. L., Spriggs, A. N., Macfie, T. S., Baden, A. L., Irwin, M. T., Wright, P. C., et al. (2016). Primate genotyping via high resolution melt analysis: rapid and reliable identification of color vision status in wild lemurs. Primates 57, 541–547. doi: 10.1007/s10329-016-0546-y
Jorgensen, A. L., Deeb, S. S., and Motulsky, A. G. (1990). Molecular genetics of X chromosome-linked color vision among populations of African and Japanese ancestry: high frequency of a shortened red pigment gene among Afro-Americans. Proc. Natl. Acad. Sci. U.S.A. 87, 6512–6516. doi: 10.1073/pnas.87.17.6512
Kawamura, S., and Kubotera, N. (2004). Ancestral loss of short wave-sensitive cone visual pigment in lorisiform prosimians, contrasting with its strict conservation in other prosimians. J. Mol. Evol. 58, 314–321. doi: 10.1007/s00239-003-2553-z
Kious, W. J., and Tilling, R. I. (1994). This Dynamic Earth : the Story of Plate Tectonics. Washington, DC: U.S. Geological Survey.
Leonhardt, S. D., Tung, J., Camden, J. B., Leal, M., and Drea, C. M. (2009). Seeing red: behavioral evidence of trichromatic color vision in strepsirrhine primates. Behav. Ecol. 20, 1–12. doi: 10.1093/beheco/arn106
Levenson, D. H., Fernandez-Duque, E., Evans, S., and Jacobs, G. H. (2007). Mutational changes in S-cone opsin genes common to both nocturnal and cathemeral Aotus monkeys. Am. J. Primatol. 69, 757–765. doi: 10.1002/ajp.20402
Li, A., and Lennie, P. (2001). Importance of color in the segmentation of variegated surfaces. J. Opt. Soc. Am. A Opt. Image Sci. Vis. 18, 1240–1251. doi: 10.1364/JOSAA.18.001240
Liebe, S., Fischer, E., Logothetis, N. K., and Rainer, G. (2009). Color and shape interactions in the recognition of natural scenes by human and monkey observers. J. Vis. 9, 14, 1–16. doi: 10.1167/9.5.14
Lucas, P. W., Darvell, B. W., Lee, P. K. D., Yuen, T. D. B., and Choong, M. F. (1998). Colour cues for leaf food selection by long-tailed macaques (Macaca fascicularis) with a new suggestion for the evolution of trichromatic colour vision. Folia Primatol. 69, 139–152. doi: 10.1159/000021576
Lucas, P. W., Dominy, N. J., Riba-Hernandez, P., Stoner, K. E., Yamashita, N., Loria-Calderon, E., et al. (2003). Evolution and function of routine trichromatic vision in primates. Evolution 57, 2636–2643. doi: 10.1111/j.0014-3820.2003.tb01506.x
Lyon, M. F. (1974). Evolution of X-chromosome inactivation in mammals. Nature 250, 651–653. doi: 10.1038/250651a0
Melin, A. D., Fedigan, L. M., Hiramatsu, C., Hiwatashi, T., Parr, N., and Kawamura, S. (2009). Fig foraging by dichromatic and Trichromatic Cebus capucinus in a tropical dry forest. Int. J. Primatol. 30, 753–775. doi: 10.1007/s10764-009-9383-9
Melin, A. D., Fedigan, L. M., Hiramatsu, C., Sendall, C. L., and Kawamura, S. (2007). Effects of colour vision phenotype on insect capture by a free-ranging population of white-faced capuchins, Cebus capucinus. Anim. Behav. 73, 205–214. doi: 10.1016/j.anbehav.2006.07.003
Melin, A. D., Kline, D. W., Hickey, C. M., and Fedigan, L. M. (2013a). Food search through the eyes of a monkey: a functional substitution approach for assessing the ecology of primate color vision. Vis. Res. 86, 87–96. doi: 10.1016/j.visres.2013.04.013
Melin, A. D., Matsushita, Y., Moritz, G. L., Dominy, N. J., and Kawamura, S. (2013b). Inferred L/M cone opsin polymorphism of ancestral tarsiers sheds dim light on the origin of anthropoid primates. Proc. Biol. Sci. 280:20130189. doi: 10.1098/rspb.2013.0189
Mollon, J. D., Bowmaker, J. K., and Jacobs, G. H. (1984). Variations of colour vision in a New World primate can be explained by polymorphism of retinal photopigments. Proc. Biol. Sci. 222, 373–399. doi: 10.1098/rspb.1984.0071
Mollon, J. D. (1989). “Tho' she kneel'd in that place where they grew…” The uses and origins of primate colour vision. J. Exp. Biol. 146, 21–38.
Moreira, L. A., de Oliveira, D. G., de Sousa, M. B., and Pessoa, D. M. (2015). Parturition signaling by visual cues in female marmosets (Callithrix jacchus). PLoS ONE 10:e0129319. doi: 10.1371/journal.pone.0129319
Morgan, M. J., Adam, A., and Mollon, J. D. (1992). Dichromats detect colour-camouflaged objects that are not detected by trichromats. Proc. Biol. Sci. 248, 291–295. doi: 10.1098/rspb.1992.0074
Moritz, G. L., Lim, N. T.-L., Neitz, M., Peichl, L., and Dominy, N. J. (2013). Expression and evolution of short wavelength sensitive opsins in colugos: a nocturnal lineage that informs debate on primate origins. Evol. Biol. 40, 542–553. doi: 10.1007/s11692-013-9230-y
Mundy, N. I., Morningstar, N. C., Baden, A. L., Fernandez-Duque, E., Davalos, V. M., and Bradley, B. J. (2016). Can colour vision re-evolve? Variation in the X-linked opsin locus of cathemeral Azara's owl monkeys (Aotus azarae azarae). Front. Zool. 13:9. doi: 10.1186/s12983-016-0139-z
Nathans, J., Piantanida, T. P., Eddy, R. L., Shows, T. B., and Hogness, D. S. (1986a). Molecular genetics of inherited variation in human color vision. Science 232, 203–210. doi: 10.1126/science.3485310
Nathans, J., Thomas, D., and Hogness, D. S. (1986b). Molecular genetics of human color vision: the genes encoding blue, green, and red pigments. Science 232, 193–202. doi: 10.1126/science.2937147
Nathans, J. (1990). Determinants of visual pigment absorbance: identification of the retinylidene Schiff's base counterion in bovine rhodopsin. Biochemistry 29, 9746–9752. doi: 10.1021/bi00493a034
Neitz, M., and Neitz, J. (2000). Molecular genetics of color vision and color vision defects. Arch. Ophthalmol. 118, 691–700. doi: 10.1001/archopht.118.5.691
Neitz, M., Neitz, J., and Jacobs, G. H. (1991). Spectral tuning of pigments underlying red-green color vision. Science 252, 971–974. doi: 10.1126/science.1903559
Newman, L. A., and Robinson, P. R. (2005). Cone visual pigments of aquatic mammals. Vis. Neurosci. 22, 873–879. doi: 10.1017/S0952523805226159
Onishi, A., Koike, S., Ida-Hosonuma, M., Imai, H., Shichida, Y., Takenaka, O., et al. (2002). Variations in long- and middle-wavelength-sensitive opsin gene loci in crab-eating monkeys. Vis. Res. 42, 281–292. doi: 10.1016/S0042-6989(01)00293-0
Onishi, A., Koike, S., Ida, M., Imai, H., Shichida, Y., Takenaka, O., et al. (1999). Dichromatism in macaque monkeys. Nature 402, 139–140.
Osorio, D., and Vorobyev, M. (1996). Colour vision as an adaptation to frugivory in primates. Proc. Biol. Sci. 263, 593–599. doi: 10.1098/rspb.1996.0089
Paramei, G. V., Bimler, D. L., and Cavonius, C. R. (1998). Effect of luminance on color perception of protanopes. Vis. Res. 38, 3397–3401. doi: 10.1016/S0042-6989(97)00454-9
Parry, J. W., Poopalasundaram, S., Bowmaker, J. K., and Hunt, D. M. (2004). A novel amino acid substitution is responsible for spectral tuning in a rodent violet-sensitive visual pigment. Biochemistry 43, 8014–8020. doi: 10.1021/bi049478w
Peichl, L., Behrmann, G., and Kroger, R. H. (2001). For whales and seals the ocean is not blue: a visual pigment loss in marine mammals. Eur. J. Neurosci. 13, 1520–1528. doi: 10.1046/j.0953-816x.2001.01533.x
Peichl, L., and Moutairou, K. (1998). Absence of short-wavelength sensitive cones in the retinae of seals (Carnivora) and African giant rats (Rodentia). Eur. J. Neurosci. 10, 2586–2594. doi: 10.1046/j.1460-9568.1998.00265.x
Peichl, L. (2005). Diversity of mammalian photoreceptor properties: adaptations to habitat and lifestyle? Anat. Rec. A Discov. Mol. Cell. Evol. Biol. 287, 1001–1012. doi: 10.1002/ar.a.20262
Perelman, P., Johnson, W. E., Roos, C., Seuanez, H. N., Horvath, J. E., Moreira, M. A., et al. (2011). A molecular phylogeny of living primates. PLoS Genet. 7:e1001342. doi: 10.1371/journal.pgen.1001342
Perini, E. S., Pessoa, V. F., and Pessoa, D. M. A. (2009). Detection of fruit by the cerrado's marmoset (Callithrix penicillata): modeling color signals for different background scenarios and ambient light intensities. J. Exp. Zool. 311a, 289–302. doi: 10.1002/jez.531
Pessoa, D. M. A., Maia, R., Ajuz, R. C. D. A., De Moraes, P. Z. P. M. R., Spyrides, M. H. C., and Pessoa, V. F. (2014). The adaptive value of primate color vision for predator detection. Am. J. Primatol. 76, 721–729. doi: 10.1002/ajp.22264
Regan, B. C., Julliot, C., Simmen, B., Vienot, F., Charles-Dominique, P., and Mollon, J. D. (2001). Fruits, foliage and the evolution of primate colour vision. Philos. Trans. R Soc. Lond. B Biol. Sci. 356, 229–283. doi: 10.1098/rstb.2000.0773
Riba-Hernandez, P., Stoner, K. E., and Osorio, D. (2004). Effect of polymorphic colour vision for fruit detection in the spider monkey Ateles geoffroyi, and its implications for the maintenance of polymorphic colour vision in platyrrhine monkeys. J. Exp. Biol. 207, 2465–2470. doi: 10.1242/jeb.01046
Saito, A., Mikami, A., Hasegawa, T., Koida, K., Terao, K., Koike, S., et al. (2003). Behavioral evidence of color vision deficiency in a protanomalia chimpanzee (Pan troglodytes). Primates 44, 171–176. doi: 10.1007/s10329-002-0017-5
Saito, A., Mikami, A., Kawamura, S., Ueno, Y., Hiramatsu, C., Widayati, K. A., et al. (2005). Advantage of dichromats over trichromats in discrimination of color-camouflaged stimuli in non-human primates. Am. J. Primatol. 67, 425–436. doi: 10.1002/ajp.20197
Saito, C. A., Da Silva Filho, M., Lee, B. B., Bowmaker, J. K., Kremers, J., and Silveira, L. C. L. (2004). Alouatta trichromatic color vision – single–unit recording from retinal ganglion cells and microspectrophotometry. Invest. Ophthalmol. Vis. Sci. 45:4276.
Sanocki, E., Lindsey, D. T., Winderickx, J., Teller, D. Y., Deeb, S. S., and Motulsky, A. G. (1993). Serine/alanine amino acid polymorphism of the L and M cone pigments: effects on Rayleigh matches among deuteranopes, protanopes and color normal observers. Vis. Res. 33, 2139–2152. doi: 10.1016/0042-6989(93)90012-L
Setchell, J. M., Charpentier, M. J. E., Abbott, K. M., Wickings, E. J., and Knapp, L. A. (2009). Is brightest best? testing the hamilton-zuk hypothesis in mandrills. Int. J. Primatol. 30, 825–844. doi: 10.1007/s10764-009-9371-0
Shaaban, S. A., and Deeb, S. S. (1998). Functional analysis of the promoters of the human red and green visual pigment genes. Invest. Ophthalmol. Vis. Sci. 39, 885–896.
Shi, Y., Radlwimmer, F. B., and Yokoyama, S. (2001). Molecular genetics and the evolution of ultraviolet vision in vertebrates. Proc. Natl. Acad. Sci. U.S.A. 98, 11731–11736. doi: 10.1073/pnas.201257398
Shu, D. G., Morris, S. C., Han, J., Zhang, Z. F., Yasui, K., Janvier, P., et al. (2003). Head and backbone of the early cambrian vertebrate haikouichthys. Nature 421, 526–529. doi: 10.1038/nature01264
Silveira, L. C., Saito, C. A., Da Silva Filho, M., Kremers, J., Bowmaker, J. K., and Lee, B. B. (2014). Alouatta trichromatic color vision: cone spectra and physiological responses studied with microspectrophotometry and single unit retinal electrophysiology. PLoS ONE 9:e113321. doi: 10.1371/journal.pone.0113321
Smallwood, P. M., Wang, Y., and Nathans, J. (2002). Role of a locus control region in the mutually exclusive expression of human red and green cone pigment genes. Proc. Natl. Acad. Sci. U.S.A. 99, 1008–1011. doi: 10.1073/pnas.022629799
Smith, A. C., Buchanan-Smith, H. M., Surridge, A. K., and Mundy, N. I. (2005). Factors affecting group spread within wild mixed-species troops of saddleback and mustached tamarins. Int. J. Primatol. 26, 337–355. doi: 10.1007/s10764-005-2928-7
Smith, A. C., Buchanan-Smith, H. M., Surridge, A. K., Osorio, D., and Mundy, N. I. (2003). The effect of colour vision status on the detection and selection of fruits by tamarins (Saguinus spp.). J. Exp. Biol. 206, 3159–3165. doi: 10.1242/jeb.00536
Smith, A. C., Surridge, A. K., Prescott, M. J., Osorio, D., Mundy, N. I., and Buchanan-Smith, H. M. (2012). Effect of colour vision status on insect prey capture efficiency of captive and wild tamarins (Saguinus spp.). Anim. Behav. 83, 479–486. doi: 10.1016/j.anbehav.2011.11.023
Stanford, C. B. (2002). Avoiding predators: expectations and evidence in primate antipredator behavior. Int. J. Primatol. 23, 741–757. doi: 10.1023/A:1015572814388
Starace, D. M., and Knox, B. E. (1998). Cloning and expression of a Xenopus short wavelength cone pigment. Exp. Eye Res. 67, 209–220. doi: 10.1006/exer.1998.0507
Sumner, P., and Mollon, J. D. (2000). Catarrhine photopigments are optimized for detecting targets against a foliage background. J. Exp. Biol. 203 (Pt 13), 1963–1986.
Sumner, P., and Mollon, J. D. (2003). Colors of primate pelage and skin: objective assessment of conspicuousness. Am. J. Primatol. 59, 67–91. doi: 10.1002/ajp.10066
Surridge, A. K., Suarez, S. S., Buchanan-Smith, H. M., and Mundy, N. I. (2005). Non-random association of opsin alleles in wild groups of red-bellied tamarins (Saguinus labiatus) and maintenance of the colour vision polymorphism. Biol. Lett. 1, 465–468. doi: 10.1098/rsbl.2005.0367
Talebi, M. G., Pope, T. R., Vogel, E. R., Neitz, M., and Dominy, N. J. (2006). Polymorphism of visual pigment genes in the muriqui (Primates, Atelidae). Mol. Ecol. 15, 551–558. doi: 10.1111/j.1365-294X.2005.02822.x
Tan, Y., Yoder, A. D., Yamashita, N., and Li, W. H. (2005). Evidence from opsin genes rejects nocturnality in ancestral primates. Proc. Natl. Acad. Sci. U.S.A. 102, 14712–14716. doi: 10.1073/pnas.0507042102
Terao, K., Mikami, A., Saito, A., Itoh, S., Ogawa, H., Takenaka, O., et al. (2005). Identification of a protanomalous chimpanzee by molecular genetic and electroretinogram analyses. Vis. Res. 45, 1225–1235. doi: 10.1016/j.visres.2004.11.016
Terborgh, J. (1983). Five New World Primates : A Study in Comparative Ecology. Princeton, NJ: Princeton University Press.
Travis, D. S., Bowmaker, J. K., and Mollon, J. D. (1988). Polymorphism of visual pigments in a callitrichid monkey. Vis. Res. 28, 481–490. doi: 10.1016/0042-6989(88)90170-8
Veilleux, C. C., and Bolnick, D. A. (2009). Opsin gene polymorphism predicts trichromacy in a cathemeral lemur. Am. J. Primatol. 71, 86–90. doi: 10.1002/ajp.20621
Veilleux, C. C., Scarry, C. J., Di Fiore, A., Kirk, E. C., Bolnick, D. A., and Lewis, R. J. (2016). Group benefit associated with polymorphic trichromacy in a Malagasy primate (Propithecus verreauxi). Sci. Rep. 6:38481. doi: 10.1038/srep38418
Verrelli, B. C., Lewis, C. M. Jr., Stone, A. C., and Perry, G. H. (2008). Different selective pressures shape the molecular evolution of color vision in chimpanzee and human populations. Mol. Biol. Evol. 25, 2735–2743. doi: 10.1093/molbev/msn220
Waitt, C., Little, A. C., Wolfensohn, S., Honess, P., Brown, A. P., Buchanan-Smith, H. M., et al. (2003). Evidence from rhesus macaques suggests that male coloration plays a role in female primate mate choice. Proc. Biol. Sci. 270, S144–S146. doi: 10.1098/rsbl.2003.0065
Wakefield, M. J., Anderson, M., Chang, E., Wei, K. J., Kaul, R., Graves, J. A., et al. (2008). Cone visual pigments of monotremes: filling the phylogenetic gap. Vis. Neurosci. 25, 257–264. doi: 10.1017/S0952523808080255
Wikler, K. C., and Rakic, P. (1990). Distribution of photoreceptor subtypes in the retina of diurnal and nocturnal primates. J. Neurosci. 10, 3390–3401.
Williams, A. J., Hunt, D. M., Bowmaker, J. K., and Mollon, J. D. (1992). The polymorphic photopigments of the marmoset: spectral tuning and genetic basis. EMBO J. 11, 2039–2045.
Xian-Guang, H., Aldridge, R. J., Siveter, D. J., Siveter, D. J., and Xiang-Hong, F. (2002). New evidence on the anatomy and phylogeny of the earliest vertebrates. Proc. Biol. Sci. 269, 1865–1869. doi: 10.1098/rspb.2002.2104
Yokoyama, S., and Shi, Y. (2000). Genetics and evolution of ultraviolet vision in vertebrates. FEBS Lett. 486, 167–172. doi: 10.1016/S0014-5793(00)02269-9
Yokoyama, S., Takenaka, N., Agnew, D. W., and Shoshani, J. (2005). Elephants and human color-blind deuteranopes have identical sets of visual pigments. Genetics 170, 335–344. doi: 10.1534/genetics.104.039511
Zhao, Z., Hewett-Emmett, D., and Li, W. H. (1998). Frequent gene conversion between human red and green opsin genes. J. Mol. Evol. 46, 494–496. doi: 10.1007/PL00013147
Keywords: trichromacy, dichromacy, visual pigments, ecology, visual opsins, evolution
Citation: Carvalho LS, Pessoa DMA, Mountford JK, Davies WIL and Hunt DM (2017) The Genetic and Evolutionary Drives behind Primate Color Vision. Front. Ecol. Evol. 5:34. doi: 10.3389/fevo.2017.00034
Received: 28 February 2017; Accepted: 05 April 2017;
Published: 26 April 2017.
Edited by:
Chuan-Chin Chiao, National Tsing Hua University, TaiwanReviewed by:
Karen Carleton, University of Maryland, College Park, USADaniel Osorio, University of Sussex, UK
Copyright © 2017 Carvalho, Pessoa, Mountford, Davies and Hunt. This is an open-access article distributed under the terms of the Creative Commons Attribution License (CC BY). The use, distribution or reproduction in other forums is permitted, provided the original author(s) or licensor are credited and that the original publication in this journal is cited, in accordance with accepted academic practice. No use, distribution or reproduction is permitted which does not comply with these terms.
*Correspondence: David M. Hunt, david.hunt@uwa.edu.au
†These authors have contributed equally to this work.